On October 11, 2019, the Kenyan Eliud Kipchoge ran the first sub-two hour marathon. The marathon requires long-term, extreme, energy expenditure. To meet that demand, Kipchoge had some unusual help: precisely calibrated support by a crew of pacers, a bicycle team supplying water and nutrition, and a pair of controversial running shoes that are said to shave a minute off the marathon time. Still, his outstanding run ranks with Usain Bolt’s record 9.58 second 100-meter race, still unbettered after 12 years, and the first 4-minute mile by Roger Bannister in 1954. In doing so, Kipchoge had to avoid the marathon runner’s nemesis: hitting the wall, also known as bonking.
The immediate source of energy for all runners, and for all muscular activity, is adenosine triphosphate, ATP. ATP is the fuel muscle needs to drive its cycles of contraction and relaxation. During that cycle, ATP is broken down to its little sister ADP (adenosine diphosphate) and phosphate, and energy is released. Subsequently, the complex biochemical pathways of energy metabolism convert carbohydrate, fat, and protein into CO2, while regenerating ATP from ADP.
The body’s fuel supplies
Fats and carbohydrate are the main fuels used to regenerate ATP. During athletic activity, protein doesn’t’ make a direct contribution. Its primary role is to perform all of the myriad molecular and cellular functions of the body, including muscle contraction.
Fats and carbohydrate exist in both stored, and immediately usable, forms. These forms can be easily interconverted, like a savings account that can be liquidated, and a cash account. The stored form of fat is mainly in the ‘triacylglycerols’, TAGs, of the adipose (“fat”) tissue. Each TAG consists of a glycerol molecule with three fatty acids esterified to it. These fatty acids are derived directly from the diet, or are produced in the liver from other foodstuffs, such as carbohydrate.
Fatty acids are released from the stored TAGs when they are needed for energy. They then circulate in blood lipid particles, and are delivered to tissues which use oxygen to generate energy. Such tissues include the heart and skeletal muscles. A small amount of TAGs are stored in muscle cells, and they can provide fatty acids for muscle activity.
Glucose is the main energy-providing carbohydrate. It circulates as “blood sugar”, which is delivered to tissues that need it. The stored form of glucose is glycogen. Glycogen is a polymer consisting entirely of glucose molecules chemically linked together. It has the same chemical composition as plant starch, and is sometimes referred to as ‘animal starch’. (The molecular linkages between glucose units in glycogen and starch are different, however.) Most of the body’s glycogen is stored in the muscles and liver. In muscle, it releases glucose when energy is needed. In the liver, it releases glucose when blood glucose levels begin to fall.
Both the sprinter Usain Bolt and Eliud Kipchoge depended on their muscle glycogen stores to perform their amazing athletic feats. But the way they used glucose was quite different. Bolt’s muscles used glucose to provide ATP by anaerobic energy metabolism (Figure 1, below) during the ten seconds that he was streaking down the track. After the race, he gulped lung-fulls of air to restore his energy stores by oxygen-requiring metabolism. At the rate he used glycogen during the sprint, he wouldn’t last long in the marathon race.
For Kipchoge, the story was different. He also depended on his muscle glycogen supply, which his well-trained body used judiciously for the duration of his two-hour marathon.
The amount of energy stored in glycogen and fat is vastly different
For a 70 kilogram male with a normal body type (not over- or underweight), the energy contained in blood glucose could sustain his energy needs for only about 15 minutes: blood glucose redistributes carbohydrate, it’s not a storage site for it. Circulating blood lipids would only sustain him for about 30 minutes. They are constantly being removed to provide energy in tissues, and replenished from the adipose tissue.
The glycogen in this man’s liver and muscles could provide energy for about 16 hours of life. In contrast, the TAGs in his adipose tissue (fat) have enough potential energy to last him 60 days, or longer. In between fat and blood carbohydrate, the protein in our bodies contains enough energy for 10 days, but we would be dead long before that if it were used as the exclusive energy source. We would expire because essential organs would fail due to a loss of their critical proteins. As a result of this, a hunger striker is more likely to die of protein depletion than of starvation.
Compared to fat, glycogen is an inefficient form of energy storage, for two reasons. First there’s the chemical nature of carbohydrate. Carbohydrate contains a lot of oxygen; its generic formula is C(H2O), repeated ‘N’ times. In other words, carbohydrate is watered carbon (in French, ‘hydrates de carbone’). For glucose, the C(H2O) formula is repeated 6 times. Human energy metabolism depends on oxidation. The amount of energy that is derived from the complete oxidation of a gram of carbohydrate (to CO2) is therefore less than it is for a gram of fatty acids: carbohydrate is already partly oxidized, fat, where the repeating formula is CH2, is not. As a result, fat requires more oxygen per gram to be converted to CO2 than carbohydrate, and yields more energy.
The second reason for the energy-inefficiency of glycogen is that it is hydrophilic — it loves water. This is a basic characteristic of carbohydrates. Fat, on the other hand, is hydrophobic — water is not attracted to it (another term for that is lipophilic, loving lipid, or fat). As stored in muscle, every gram of glycogen attracts, and binds to, 3 or 4 grams of water. This reduces the energy density even more. So, while dry carbohydrate (sugar in the bowl) contains about 45% as much energy, per gram, as fat, in the body that ratio for glycogen relative to TAGs is closer to 13%. For the fit young male described above, converting all of the energy contained by his adipose tissue into glycogen would add something like 125 kilograms (270 pounds) to his weight.
The primary advantage of glycogen for energy storage is that it provides energy quickly, for example, to Usain Bolt’s pounding leg muscles. The primary advantage of TAGs (fat) is that they can store enormous amounts of energy with relatively little weight. There’s an evolutionary advantage to that; many animals in the wild, and until relatively recently, humans as well, depend on the energy content of TAGs to survive food deprivation.
Although the contributions of carbohydrate and fat to energy metabolism have different characteristics, they are intimately linked. A closer examination of the energy metabolism fueled by carbohydrates and by fats shows why.
The energy economy: sugar
The diagram below (Figure 1) outlines how carbohydrate is used for energy production in a working muscle (or any tissue). The sources of glucose inside the muscle cell are blood glucose and glycogen. Blood delivers glucose to muscle cells at a modest rate, fast enough to replenish the muscle’s supply of carbohydrate after a race, but not fast enough to power a marathon by itself.
As soon the starter’s gun fires, metabolic and hormonal signals start the breakdown of glycogen to glucose inside the muscle cell. This is true for both Usain Bolt and Eliud Kipchoge. The glucose released from glycogen in a muscle cell stays in that cell; there’s no mechanism for replenishing blood glucose, for example, from muscle glucose. And a muscle cell that runs out of carbohydrate cannot get it from another muscle cell. It’s a local resource.
Glucose enters anaerobic (oxygen-independent) energy metabolism, glycolysis, in which about 20% of its potential energy is released in the form of ATP regenerated from ADP. Some of the glucose is converted to lactate, which is the end product of anaerobic energy metabolism (like Usain Bolt’s).
In the distance runner, much of the product of glycolysis is fed into aerobic (oxygen-requiring) energy metabolism (the green circle). In that scheme, oxygen is used to completely convert the input materials to CO2, and the energy released by this oxidation is used to regenerate ATP. (Oxidative metabolism comprises the citrate cycle and oxidative phosphorylation. Fortunately, an understanding of these complex systems is not required to follow the logic of this description.)
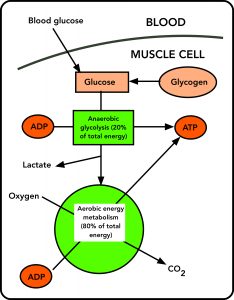
For the marathon runner, blood glucose plays an important role in this scheme. Although it cannot supply glucose fast enough to power the whole of the race, it does provide extra glucose, as the runner takes in nutrition during the race. This extra glucose extends the distance the runner can go at speed, as will be explained, and it prevents a catastrophic drop in blood glucose levels, which could cause the runner to black out.
The energy economy: fat
The role of fat in providing energy is a little more complicated, but it is intimately connected to the sugar pathway. Fat, in the form of TAGs, releases fatty acids in response to the same hormonal signals that stimulate glycogen breakdown (the “anti-insulin” hormone, glucagon, which responds to low blood sugar, and the “fight or flight” hormone adrenaline, which responds to fear or challenge). And these fatty acids are also a fuel for ATP resynthesis. Some TAGs are stored in muscle cells, but most of the fatty acids provided to working muscle cells come from outside of it, from the adipose tissue.
The initial stage of fat use is the partial breakdown of the long-chain fatty acids through a sequence of reactions that yield about 25-30% of the energy of the fatty acids. This requires oxygen, and produces ATP. The products of this first step are fragments containing just 2 carbon atoms, called acetyl-Coenzyme A. (Again the biochemical details aren’t necessary.)
Further oxidation of the 2-carbon fragments produced in stage one depends on the carbohydrate metabolism of the muscle cell. It’s this that ties together carbohydrate and fat-driven energetics.
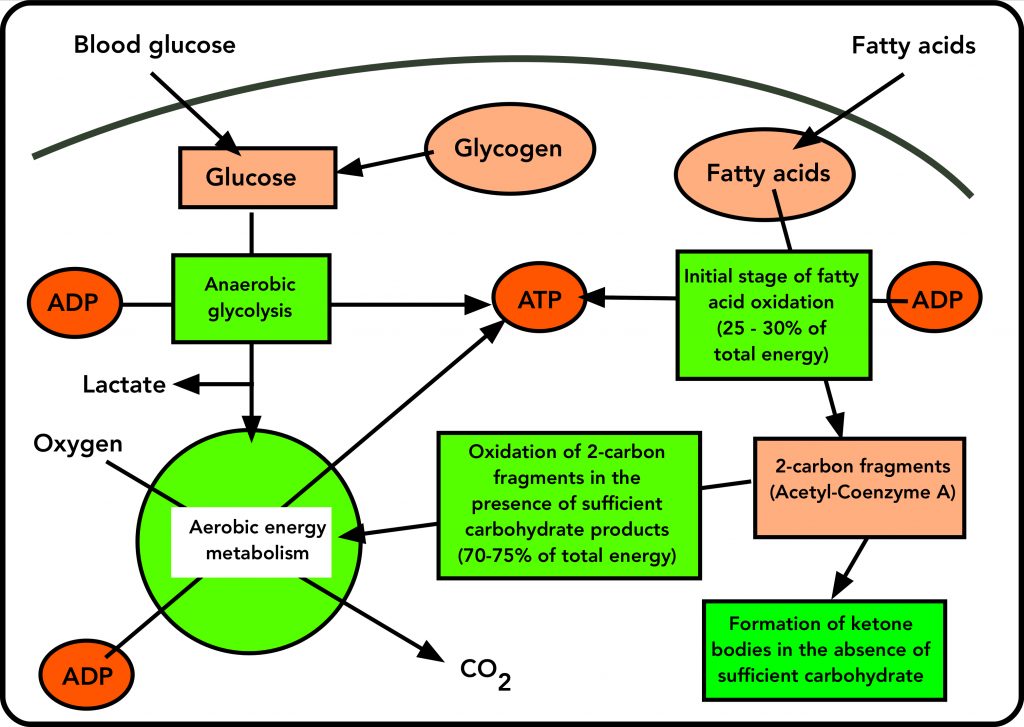
In a muscle cell that has sufficient carbohydrate from anaerobic glycolysis, the 2-carbon fragment acetyl-Coenzyme A enters the same aerobic (oxygen-dependent) energy metabolism (the green circle in Figures 1 and 2) as the products of glycolysis. There, they are converted to CO2, and this oxidation powers the resynthesis of ATP from ADP.
There’s a critical difference between the entry of carbohydrate and fat into aerobic energy metabolism. On the one hand, products of glycolysis (from glucose) enter the aerobic energy metabolism scheme directly, since they provide the key priming molecule for it. But the 2-carbon product of fat breakdown (acetyl-Coenzyme A) can only enter the scheme if there’s enough carbohydrate to keep the cycle of oxidative energy metabolism going. This means that Acetyl-coenzyme A cannot drive aerobic energy metabolism by itself, since it doesn’t provide the priming molecule required. Instead, it depends on carbohydrate products to prime the aerobic energy cycle. This is reflected in the old adage “Fat burns in the carbohydrate fire”. (For the curious, that priming molecules is oxaloacetate, which is produced by carbohydrate catabolism, but not by fatty acid oxidation.)
So aerobic energy metabolism from fat dwindles when there’s a shortage of the products of glycolysis (which are derived from glucose). When this happens, the product of fatty acid breakdown (acetyl-coenzyme A) enters the oxidative energy cycle at an ever-decreasing rate. Unable to enter the oxidative scheme, some of the 2-carbon fragments condense into ketone bodies: beta-hydroxybutyrate and acetoacetate, familiar to serious runners and followers of low-carbohydrate diets. (The third ketone body is acetone, which may show up as a bad breath.) These leave the muscle through the blood.
Is fat necessary to run a marathon?
Can glycogen alone provide the energy for a marathon? Scientists who study the sport conclude that it can’t (1). Glycogen-loaded muscle (serious runners know what that is) may come closer but it still fall short. Essentially all of the glycogen in the running muscles is used up during a marathon, and even elite runner depend partly on energy provided by fat oxidation (2, 3) This is also true for other endurance sports, like cycling (4).
Runners who complete the marathon have trained to consume their vital muscle glycogen at a measured rate, so that it doesn’t disappear before the end of the race. Fat will not run out, but carbohydrate may. By the end of the marathon, elite runners will have obtained about 60% of their energy from carbohydrate, which was originally glycogen and blood glucose, and 40% from the oxidation of fat, originally in TAGs (1).
Some of the fatty acids liberated from TAGs to provide energy during the marathon end up being converted to ketone bodies, even in successful (i.e. finishing) runners. In one study, the beta-hydroxybutyrate levels in the blood of a group of runners went up 3.7 -fold by the end of the marathon, and acetoacetate, 9-fold (5). This is an indication that there wasn’t enough carbohydrate coursing through aerobic energy metabolism to accept all of the fatty acid breakdown products.
The fuel mix in the marathon
The marathon represents the limit of human ability to run at a fast pace (and the elite runner is fast: try running 12 miles an hour, and then imagine doing it for 26 miles). There is a relationship between how fast the runner goes, how much oxygen her system delivers to her muscles, and the amount of carbohydrate she consumes. As running speed increases, oxygen consumption goes up. As oxygen consumption goes up, the ratio of carbohydrate to fat in the fuel mix goes up as well, as carbohydrate delivers more energy, per volume of oxygen consumed, than fat. This is illustrated in the following graph:
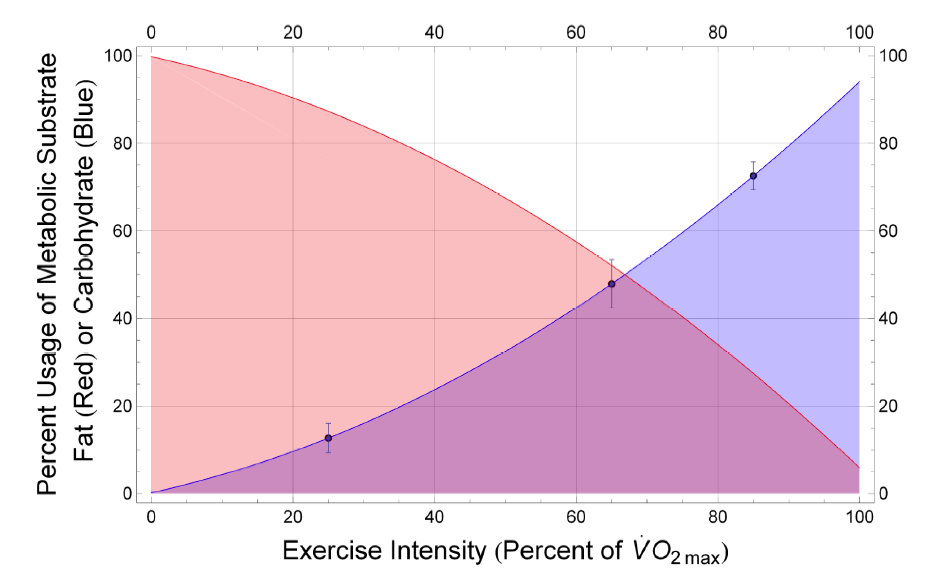
To complete the race in the fastest time possible for her, she must conserve glycogen, yet use almost all of it up by the end. One scientist has concluded that the best fuel economy for an endurance race is achieved at a constant level of exertion (1).
Blood glucose doesn’t change much during a successful marathon, and hypoglycemia (low blood sugar) doesn’t occur if there’s enough carbohydrate being taken in during the race (6). However, blood lactate does go up, which indicates that the runner is getting some energy from anaerobic (oxygen-independent) glycolysis, which produces lactate (Figure 1). In one race, a group of faster marathon runners had significantly higher blood lactate levels than a group of slower runners, indicating a greater utilization of anaerobic glycolysis (7, 8).
There is a growing interest in the role of ketone bodies in athletic performance, particularly distance running. In one study, the performance of (non-elite) marathon runners on a keto diet was good up to about 70% of maximum oxygen capacity; after that, keto-adapted runners didn’t go as fast for a given oxygen intake (9). Perhaps surprisingly, the level of muscle glycogen, and dependence on it during a race, was the same for those on a keto diet as for those eating conventional levels of carbohydrate (10). But a keto diet did increase the utilization of fatty acids for energy. Taken together, these results suggest that a keto diet has little impact on the performance of the average runner. But most elite runners eat a balanced diet, with a pre-race emphasis on ‘glycogen loading’, to optimize their performance.
Blood glucose, while it doesn’t provide enough of an energy pipeline to sustain the marathon runner, appears to be important for the overall energy economy of the marathoner. In trained cyclists, whose level of performance is similar to that of marathon runners, the intake of glucose during the race delayed exhaustion for an hour after muscle glycogen was depleted (4). A comparable group that didn’t take in glucose during the race was exhausted, and had to stop, when glycogen ran out. Carbohydrate intake during a race, which results in a higher blood glucose level, thus seems to provide important extra fuel.
Hitting the wall
For non-elite marathoners, success is spelled “F-I-N-I-S-H”. Many don’t. It has been stated that more than 40% of the starters in a typical marathon “hit the wall”, and then may or may not be able to complete the distance (11). Hitting the wall, or “bonking”, is the marathon runner’s nemesis. There’s a feeling of complete energy depletion, dizziness, inability to focus, and an inclination to walk or even sit down. The potential causes are several, including dehydration and salt imbalance (12), but the most common is also the hardest to counter: running out of energy.
How can a runner run out of energy? There’s enough fat in his or her body to run several marathons. The problem is not the quantity of energy; it’s the rate that it can be used. Fat can be the major source of energy in ultra-long races for runners who are highly trained to utilize it. But for the average marathon runner, or for the elite runner who is moving at a five-minute-mile pace, no more available glycogen means no more ability to run, and the bonk. That happens characteristically at around mile 20, which is about as far as glycogen alone will take the runner.
Depletion of glycogen, and the shut down of carbohydrate metabolism, affects energy levels in three ways. With a shortage of glucose and glycogen, the energy produced by anaerobic glycolysis, which generates lactate, is shut down. Furthermore, the aerobic metabolism of carbohydrate, which is responsible for about 80% of its energy, dwindles. And the shortage of carbohydrate means that the 2-carbon fragments resulting from the breakdown of fatty acids cannot enter aerobic metabolism. Instead, as explained in Figurte 2, those fragments are instead converted to ketone bodies. The result is an energy crisis.
In a laboratory situation that mirrors the marathoner’s bonk, athletes were told to pump an exercise bicycle with one leg to exhaustion, while the other leg rested. Exhaustion occurred typically after 2 hours (13). At that point, muscle glycogen was exhausted in the working leg, while the other leg still had its original level of glycogen. This reflects the fact that the glycogen in a particular muscle is limited to energy production in that muscle. It cannot supply other muscles, nor blood glucose.
There’s a significant amount of opinion expressed on the Internet that premature exhaustion in a race is due to hypoglycemia, low blood sugar. While blood glucose may fall in the latter parts of a race, finishing marathoners do not show signs of hypoglycemia (14). I’m not aware of studies of the blood glucose levels of runners who have hit the wall, but in studies where athletes are pushed to exhaustion by intense exercise, their blood glucose remains fairly close to normal (13).
Go to Latest Posts
Sources
- Rapoport, B. I. 2010. Metabolic factors limiting performance in marathon runners. PLoS Comput Biol 6:e1000960.
- Sherman, W. M., D. L. Costill, W. J. Fink, F. C. Hagerman, L. E. Armstrong, and T. F. Murray. 1983. Effect of a 42.2-km footrace and subsequent rest or exercise on muscle glycogen and enzymes. J. Appl. Physiol. Respir. Environ. Exerc. Physiol. 55:1219-1224.
- Asp, S., T. Rohde, and E. A. Richter. 1997. Impaired muscle glycogen resynthesis after a marathon is not caused by decreased muscle GLUT-4 content. J Appl Physiol 83:1482-1485.
- Coyle, E. F., A. R. Coggan, M. K. Hemmert, and J. L. Ivy. 1986. Muscle glycogen utilization during prolonged strenuous exercise when fed carbohydrate. J. Appl. Physiol. (1985) 61:165-172.
- Scheele, K., W. Herzog, G. Ritthaler, A. Wirth, and H. Weicker. 1979. Metabolic adaptation to prolonged exercise. Eur J Appl Physiol Occup Physiol 41:101-108.
- Noakes, T. D., E. V. Lambert, M. I. Lambert, P. S. McArthur, K. H. Myburgh, and A. J. Benade. 1988. Carbohydrate ingestion and muscle glycogen depletion during marathon and ultramarathon racing. Eur J Appl Physiol Occup Physiol 57:482-489.
- O’Brien, M., C. A. Viguie, R. S. Mazzeo, and G. A. Brooks. 1993. Carbohydrate dependence during marathon running. Medicine and Science in Sports and Exercise 25:1009-1017.
- Hoyt, R. W., J. M. Egler, and T. Asakura. 1984. Relationship between uphill running performance at altitude and blood metabolite levels. Clin Physiol Biochem 2:192-197.
- Shaw, D. M., F. Merien, A. Braakhuis, E. D. Maunder, and D. K. Dulson. 2019. Effect of a Ketogenic Diet on Submaximal Exercise Capacity and Efficiency in Runners. Med Sci Sports Exerc 51:2135-2146.
- Volek, J. S., D. J. Freidenreich, C. Saenz, L. J. Kunces, B. C. Creighton, J. M. Bartley, P. M. Davitt, et al.2016. Metabolic characteristics of keto-adapted ultra-endurance runners. Metabolism 65:100-110.
- Buman, M. P., B. W. Brewer, A. E. Cortnelius, J. L. Van Raalte, and A. J. Petipas. 2008. Hitting the wall in the marathon: Phenomenological characteristics and associations with expectancy, gender, and running history. Psychology of Sport and Exercise 9:177-190.
- Kratz, A., A. J. Siegel, J. G. Verbalis, M. M. Adner, T. Shirey, E. Lee-Lewandrowski, and K. B. Lewandrowski. 2005. Sodium status of collapsed marathon runners. Arch Pathol Lab Med 129:227-230.
- Casey, A., A. H. Short, E. Hultman, and P. L. Greenhaff. 1995. Glycogen resynthesis in human muscle fibre types following exercise-induced glycogen depletion. J. Physiol. 483 ( Pt 1):265-271.
- Kratz, A., K. B. Lewandrowski, A. J. Siegel, K. Y. Chun, J. G. Flood, E. M. Van Cott, and E. Lee-Lewandrowski. 2002. Effect of marathon running on hematologic and biochemical laboratory parameters, including cardiac markers. Am J Clin Pathol 118:856-863.